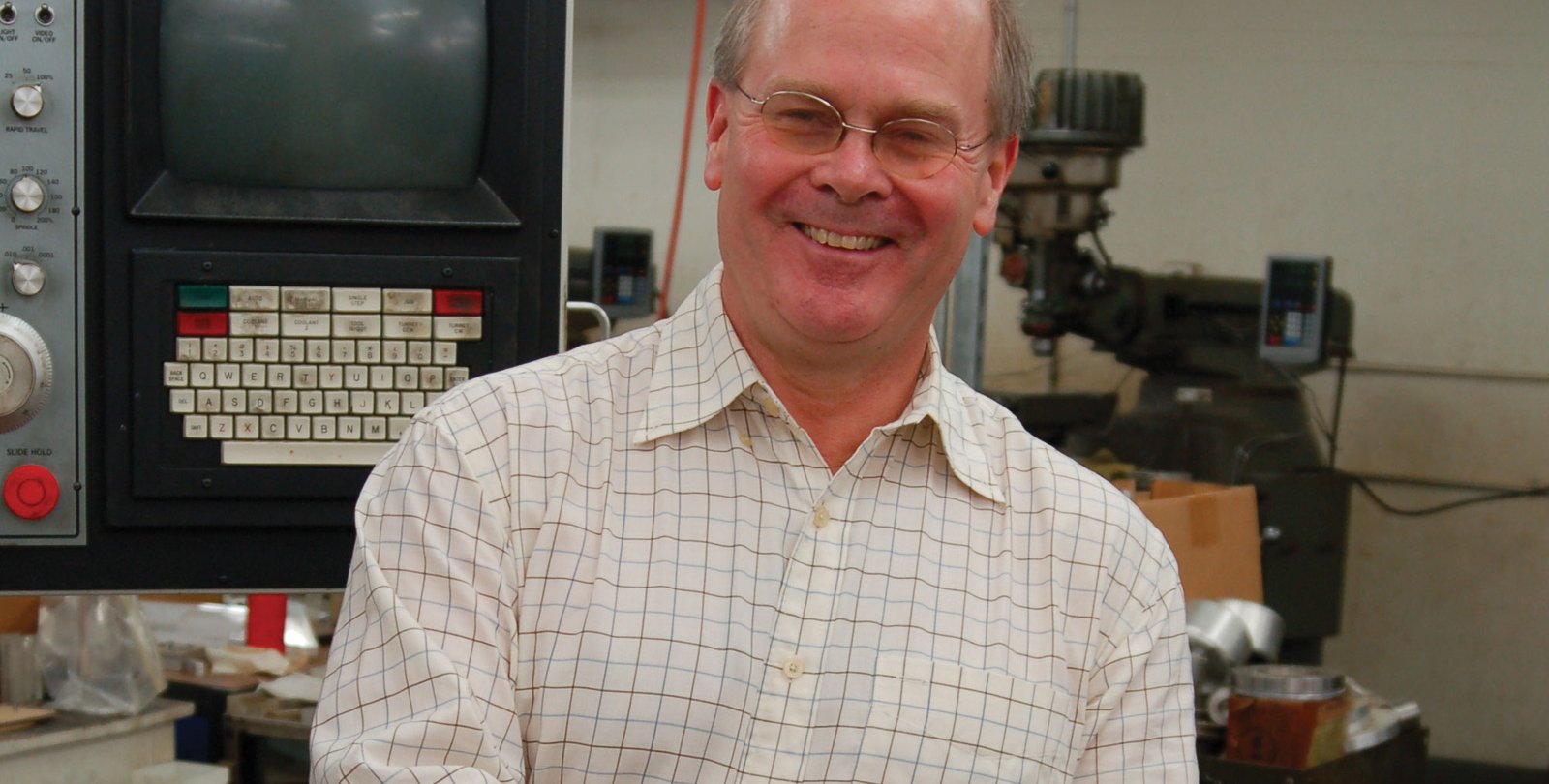
The Next Step: Stimulating Electrode Array Assists Paraplegic Man to Stand and to Move Legs Voluntarily
There is a long history of positive accidental results in experimental science. However, when a paralyzed man regains some of his voluntary movements in the accidental result, there is some humanitarian icing on the cake.
Our Caltech team, which includes Yu-Chong Tai, Professor of Electrical Engineering and Mechanical Engineering, in collaboration with Professor Reggie Edgerton at the University of California, Los Angeles, and Professor Susan Harkema at the University of Louisville, has used an epidural stimulating electrode array to assist a 25-year-old paralyzed male athlete to stand, to step on a treadmill with assistance, and, over time, to regain voluntary movements of his limbs. Using a combination of experimentation, computational models of the array and spinal cord, and machine-learning algorithms, we are now trying to optimize the stimulation pattern to achieve the best effects and to improve the design of the electrode array. Further advances in the technology should lead to better control of the stepping and standing processes. More importantly, we hope to better understand and advance our ability to help patients regain voluntary control over their once-paralyzed limbs. We are continuing our experiments to try to duplicate these results on other patients, and our initial results on our second patient are very encouraging.
While my primary research focus has been robotics and mechanical systems, for over a decade I have been investigating and developing adaptive electrode arrays that can interface computers to damaged nervous systems. For nearly a decade, my group collaborated with Caltech's Professor Richard Anderson in the area of neural prostheses, which are direct brain interfaces that allow control of electromechanical devices by thoughts alone through the use of surgically implanted electrode arrays and associated computer algorithms. Eight years ago, my group started working with Dr. V. Reggie Edgerton, Professor of Neuroscience at UCLA, who has worked on spinal-cord injuries for many years and, in particular, on the use of epidural stimulation as a potential therapy. In epidural stimulation, the stimulating electrode is not directly implanted into the spinal tissue. Such a placement would require opening the dura (a thick sheath surrounding the central nervous system), increasing the possible chances for a life-threatening infection. Instead, epidural stimulating electrodes are placed in the epidural space that exists between the interior walls of the vertebra and the dura. We were able to bring some new ideas to Dr. Edgerton's extensive experience in this area. We introduced the idea that an epidural electrode array, in contrast to the small number of wire electrodes used at the time, should significantly improve our ability to more precisely control the stimulation process, leading to better outcomes.
To build these arrays at a small enough scale for experiments in rodent models, we engaged Professor Yu-Chong Tai's group to develop miniature micro-fabricated electrode array systems. While the development of micro-fabricated array technology is still ongoing, our initial animal results with these arrays were impressive enough to convince the National Institutes of Health and the Food and Drug Administration to allow us to implant five humans with epidural stimulating arrays.
Our first patient, Rob Summers, was a top-tier college athlete who was severely injured when he was a hit by an automobile. Rob had been in a wheelchair for nearly three years at the time we implanted a 16-channel electrode array over the lower portion of his spine. Within three weeks after the implantation, the physical therapy team at the University of Louisville had him standing independently with the aide of stimulation. Perhaps more importantly, after about five months of daily stimulation and exercise, Rob started reporting increased control over his bladder and bowel function. In many spinal-cord injuries, not only is the ability to control muscles lost, but the autonomic nervous system, which controls bladder, bowel, breathing, blood pressure, etc., is severely damaged. Amazingly, starting at eight months after implantation, Rob regained the ability to voluntarily command movements of his lower limbs. At first, he could wiggle his toes, then he could move his knees, and finally he could flex his hips. These voluntary movements required the stimulating electrode array. Very recently, however, 21 months after the implantation, he made his first voluntary movement without the aid of the stimulator. Over this period, Rob has regained essentially full control of his bladder and bowel, and has significantly improved cardiovascular health, as well as increased sensation below the level of his spinal injury. We never anticipated these collateral benefits to our human subject!
In early August 2011, we implanted our second human subject. Already, this subject is following the same recovery trajectory we saw in Rob, and in several areas, he is recovering even more quickly. So, it appears that our results can be replicated.
One of my main research objectives is to develop the theory and algorithms that will help us better optimize the stimulating patterns that we apply to the array. At the moment, we find the "optimal" stimulating parameters by brute-force experiment. As our ability to fabricate and deploy arrays with greater numbers of electrodes increases, it is practically impossible to explore the vast space of possible stimulating parameters by brute force alone. To address this problem, my group is pursuing two approaches. First, we are developing a computational model where the entire bio-electrophysics of the electrode array stimulation process is captured. We are building increasingly accurate geometrical models of the spinal cord using high-field-strength MRI images obtained in Caltech's Broad Imaging Center. The electrical properties of the different spinal tissue components are superimposed on the geometries extracted from the MRI model. Next, we build models of the electrode arrays with their resistive and capacitor properties. Then we can simulate how the electric fields penetrate the tissue, and the probabilities that the fields excite or facilitate the operation of different types of neurons. A number of questions can be addressed with the computational model. How do we optimize the electrode stimulation pattern that we apply? Can we use the stimulation to help us optimize the array design itself? Do we want more electrodes or fewer electrodes over time? Should the electrodes be bigger or smaller? Should they be placed toward the side of the cord or more toward the middle? What is the optimal shape(s) of each electrode? All of these questions can be considered with the simulation system.
Because no model can be perfectly accurate, in our second approach to this problem, we are working with Professor Andreas Krause to develop a machine-learning algorithm to optimize the stimuli. In this process, we apply an electrode stimulation pattern and then watch how the animal or human responds. Based on the response, we use an algorithm that figures out what stimuli should be tried next. It's excellent at starting to build an internal mental model of how the arrays work and then trying to optimize and explore different stimuli over time. Since every person's injury and subsequent response to stimulation is bound to be a little bit different, such an approach is necessary to fine-tune this therapy for each patient. Also, as a patient recovers, the necessary stimuli will change over time. We did not have the learning algorithm ready for patient number one, but we plan to use it for patient number three. Ideally, in the future there will be a smart algorithm monitoring the patient's response on an ongoing basis, perfecting the stimuli to meet his or her evolving needs. Right now, clinicians monitor and adjust the stimuli based on observations and intuition. This is great on a one-patient one-clinic basis, but how do we replicate it to more clinics and patients? Clearly, algorithms embedded into the stimulating infrastructure can help with this scaling problem.
Physical training must be carried out in conjunction with the epidural stimulation to achieve a successful outcome. The lumbosacral locomotor circuitry must adapt to the new command signals provided by the stimulating array. In our animal work, we have developed specialized robotic devices to administer and monitor the physical therapy. We have also started to build equipment that can be used by our patients in their home environment. We are now considering automation of the type that we have developed for our animal work in order to allow this human therapy to be deployed on a larger scale.
This is a very exciting area of research, and many questions still remain. In no way have we come close to finding a "cure" for spinal-cord injury. But our initial results are promising, giving us the hope that this therapy can have a positive impact on a wide range of the spinal-cord injured. Can we accelerate the process and make it work in a variety of patients? That is our next challenge. If we can understand the method better and understand the neurobiology better, we can then try to optimize the technology and its delivery for a wider range of people with different kinds of injuries. There are also tantalizing hints that this approach may also provide some benefit to other debilitating conditions, such as stroke or Parkinson's disease. In the long run, a biological approach (stem cells, neural tissue implants, or genetic manipulation of the neural regrowth mechanisms) is clearly the preferred solution. However, such a solution is very likely to benefit from, and perhaps even require, the approach that our Caltech, UCLA, and Louisville team has been developing.
Joel W. Burdick is Professor of Mechanical Engineering and Bioengineering.